The purpose of this post is to re-examine the quasi electric and quasi magnetic fields using the seminal work of Le Blonde and Le Ballac (1973).
A second part of this post is entitled The Character of Quasi Static Fields,
Introduction
Quasi static fields cover the behaviour of fields emitted from slowly moving charges, and therefore covers behaviour between the static and propagating electromagnetic fields. Quasi static fields are defined as fields with the “absence of propagating waves”. In other words non-static (i.e. not static charges) fields and that are not transmitted (i.e not self-sustaining electromagnetic fields, called photons)
An excellent treatment of quasi static electric and magnetic fields can be found in Melcher (1998). However, this text reflects the view that quasi static equations were only useful “approximations” for engineering and does not saying anything qualitatively new about these fields. LeBlonde and Le Ballac (1973) treated quasi static electric and magnetic fields as first class theoretical entities and enabled a clearer theoretical analysis of their behaviour. This work enables a more consistent and through understanding of the relationship between static, quasi-static and propagating electromagnetic fields.
The history of physics runs along the lines that Newton discovered the behaviour of (1) slow moving bodies, including gravity. Maxwell then discovered the behaviour of (2) luminal electromagnetic fields. Einstein then discovered the behaviour of (3) luminal bodies, which led to the theory of spacetime. As Rousseaux (2013) points out these three major discoveries left out the behaviour of slow moving fields. that Levy Le Blonde and Le Ballac (1973) discovered the behaviour of (4) slow moving, or quasi static fields, as a missing gap in field theory. So there is in fact a two dimensional matrix of slow and fast moving bodies and slow and fast moving fields that have been discovered.
Fields emitted from slow moving bodies are actually the most common form of fields inside the three common forms of matter: liquids, solids and gases. Electromagnetic fields being transmitted from solar plasma at the speed of light through the vacuum of space, or electronic ariels are exceptional nature.
Quasi static fields is the study of fields arising from slow moving matter. A scientist investigating fields in the brain should sensibly start with the theory of quasi static fields emitted from slow moving ions. Propagating electromagnetic fields (i.e. light) is not commonly found in the brain. From this it follows consciousness is probably the result of quasi static fields which shows that Levy-LeBlonde and Le Ballac’s treatment is not complete because it does not provide a complete interpretation of the reality of slow moving fields in relation to consciousness. Understanding quasi static fields and consciousness in the brain has been the thesis of these posts.
Maxwell’s Electromagnetic Laws
When Maxwell first introduced his equations he distinguished between electric and magnetic flux and electric and magnetic force.
Heaviside (1885) took Maxwell’s equations and used vectors (E,H,B,D) to define two forces and two fluxes:-
* E – the electric field intensity (force)
* H – the magnetic field intensity (force)
* B – the magnetic flux density (flux)
* D/j – the electric flux/current density (flux)
According to classical electromagnetic theory (see below). The curl of electric force intensity (E) is produced by the change in magnetic flux density (B). The curl of magnetic force (H) is produced by the sum of the change in the electric current displacement (D) and the current density (j). The divergence of the magnetic flux density (B) is produced by the non-divergent magnetic curl (closed curl). The displacement of electric flux (D) is produced by the the charge density (ρ) .
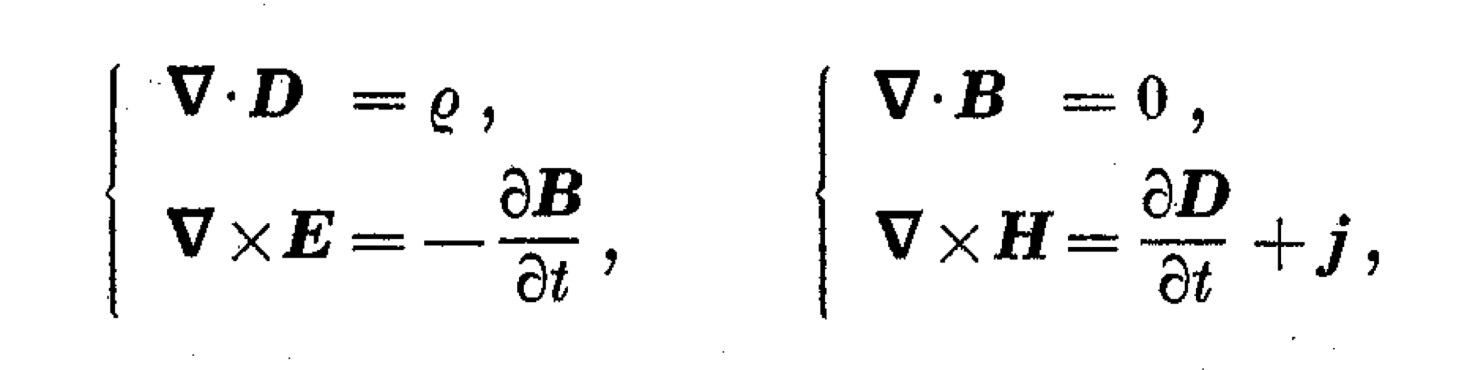
Showing Maxwell’s equations in the relativistic limit using both flux and force (Le Blonde and Le Ballac 1973).
The calculus notation on Maxwell equations can also be simplified to the following (Rousseaux 2005) :-
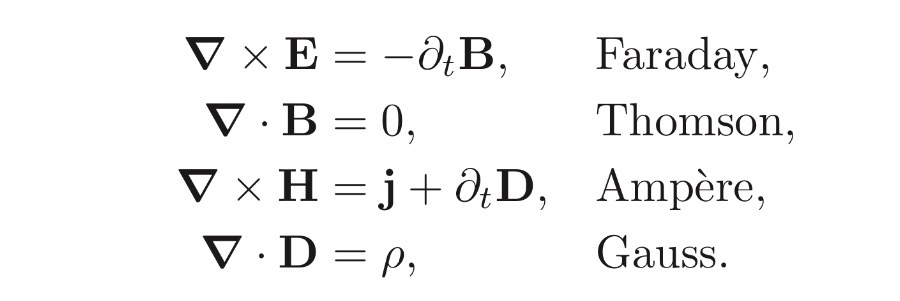
Showing a simplified version of Maxwells Equations (Rousseaux 2013).
As a result of Einstein, the speed of electric and magnetic fields was commonly determined as the speed of light, and this speed would slow down in a medium due to the (electric) permeability (ε) and (magnetic) permittivity (µ). In a vacuum it these values are determined to be 1 and are denoted as ε0 and µ0.
The relationship between forces (E and H) and (D and B) flux are very commonly “simplified” in the modern versions of Maxwell’s equations above by assuming permeability (ε) and permittivity (µ) are 1. So that the assumptions below are made:-

When electric permeability (ε) and magnetic permittivity (µ) are included in modern conventions, the equations show how permeability and permittivity modify the equation. The curl of magnetic force (xH) is modified by the magnetic permittivity (µ) becomes the curl of the electric force (xB). The displacement of electric flux (.D) is modified by the electric permittivity (ε) and becomes the displacement of electric force (.E).
In the relativistic limit, where ε0 and µ0 are included the equations look as follows:-
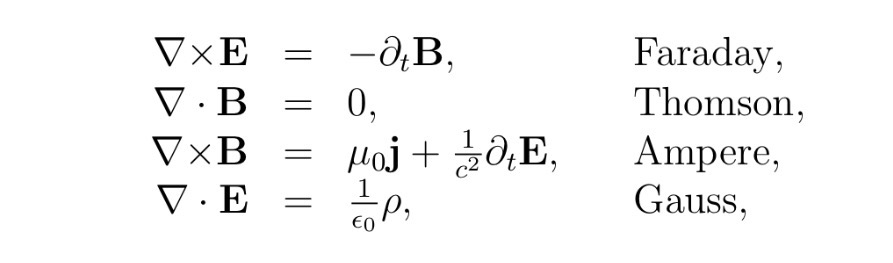
Showing a Maxwells Equations including ε0 and µ0 (Rousseaux 2013).
Slow. moving charges
The expressions in Maxwell’s equations are fully utilised when electromagnetic fields are transmitted from a source. The electromagnetic configuration of transmitting fields was imagined by Maxwell as a feedback loop where energy oscillated between electric and magnetic forces and flux. Later Hertz discovered how a configuration of rapidly moving charges related the transmission of electromagnetic waves. Transmitting electromagnetic waves are relativistic (i.e.the speed of light) and time retardation (i.e. light transmission speed) between the transmitter and receiver need to be taken into account.
A different configuration involving slowly moving charges creates emitting (not transmitting) electric and magnetic fields and does not utilise all the equations in Maxwells equations because the feedback loop is not created. Configurations include motors and dynamos, as well as oscillating charges in brains. This simplification of Maxwells Equations is used by electronic engineers and is known as quasi statics (QE) approximations. The oldest reference to quasi statics is the book by Woodson and Melcher in 1968 (Rousseaux 2013). In treatment of EEG and MEG these limits have been used to simplify equations (Nunez 2006 – appendix B). These engineering simplifications say nothing about the nature of the electromagnetic fields, just that they simplify Maxwells Equations
Quasi-statics is defined as where “the system is small compared with the electromagnetic wavelength associated with the dominant time scale of the problem.” (Jackson 1999). A large range of commonly used electrical equipment fall within the quasi-static regime, including motors, transformers and microelectronics. Quasi-statics are traditionally taught as a simplification of the Maxwell equations, used to help engineers with their calculations on such electronic equipment.
Le Blonde and Le Bellac (1973) showed in quasistatics there are two well defined mutually exclusive (non-relativistic) limits to electrodynamics. One is called the the electric limit and the other the magnetic limit. These limits involve low velocity charges. Where the magnetic field is dominant this leads to the magnetic limit. Where the electric field is dominant this leads to the electric limit. (De Montigny 2005). In addition to the electric and magnetic limits there is a more complex hybrid limit known and the Darwin limit, which utilises more, but not all of Maxwells Equations.
In engineering the following approximations are made using E and B:- (Haus 2000, Castellenos 1998):-
- Electro Quasi Statics (EQS) simplify Maxwell’s equations by neglecting the time-derivative of the magnetic field (∂tB) in Faraday’s law : ∇ × E ≃ 0
- Magneto Quasi Statics (MQS) simplify Maxwell’s equations by neglecting the time-derivative displacement current (∂tE) in Ampere ‘s law : ∇ × B ≃ μ0J
- Darwin’s Approximation simplifies Maxwell’s equations by neglecting “a part of” ∂tE in Ampere law.
Note: EQS and MQS are mutually exclusive limits (Castellenos 1998)
For the engineers looking to simplify Maxwells equations for charges moving at low speeds, the Galilean theory of instantaneous interaction was used because it simplified the calculations point of view. However Le Blonde and Le Bellac (1973) discovered that Galilean Electromagnetism was not just an approximation but real for some interactions. A feature of quasistatics is instantaneous interaction (i.e Galilean relativity) at a distance (Larson 2006). The fields are propagated instantaneously (i.e c → ∞) so there is an absence of time-retardation.
The relationship between different types of fields can be seen in the diagram below. This is defined using a dimensionless parameter α which defines the quasistatic region. Where the wavelength λ = c/T, then the quasistatic limit α ≪ 1 corresponds to L ≪ λ, i.e., the wavelengths are much larger than the characteristic length of interest. Where σ = order of magnitude.
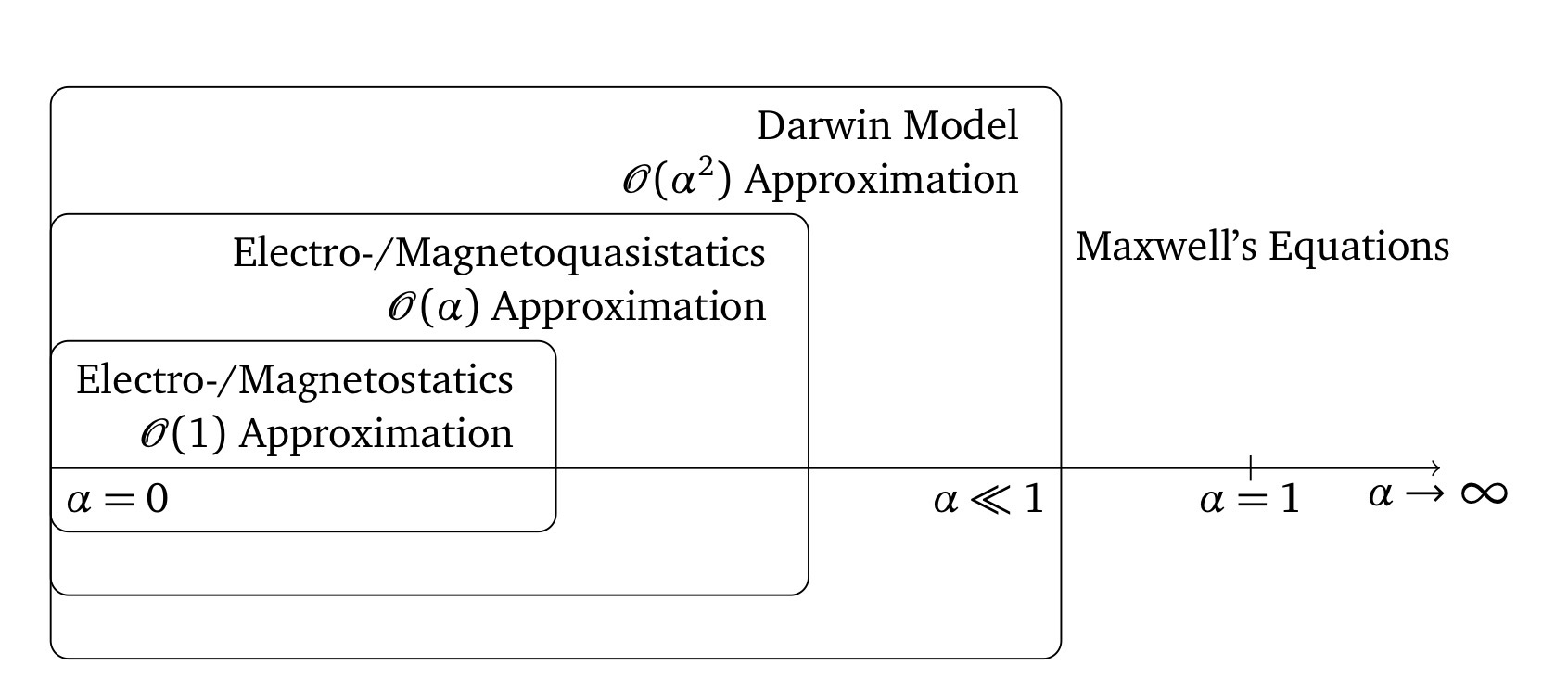
Diagram showing the relations between the various electromagnetic limits (Traub 2014).
The Electric Limit
In the electric limit Isolated electric charges move with low velocities. In the electric limit the motion of an electric field (or its time variation) produces a magnetic field, while a time varying magnetic field does not produce an electric field (Le Blonde and Le Bellac 1973). In the electric limit a magnetic field exists but has no effect (Rousseaux 2006).
These equations describe where isolated charges move with slow velocity.
Quasi Electric Maxwell equations (Le Blonde and Le Ballac 1973).
Effectively an electric field (Ee) is generated by electric sources and a magnetic field (Be) that are generated by an electric source. In this limit the magnetic field is not time varying (− ∂tB) and will not create an electric field (∇ × Ee) to couple back onto a magnetic field (Rousseaux 2006).
Faraday’s law of induction does not work. Thus Faradays law of V x E = -∂B/∂t is now V x EE = 0. In the electric limit the electric curl has 0 field in Faraday’s Law.
From an engineering perspective the electric limit is an approximation. “the EQS regime is a good approximation in the interior and vicinity of dielectrics, which might exhibit some losses which takes displacement currents are taken into account and neglects Faradays law” (Kutrtz 2010).
Importantly the magnetic field is incorrectly concluded not to exist by engineers because it does not have any effect. “In the EQS regime, there is no magnetic induction. The EQS regime includes capacitive but not inductive effects because the electric field does not produce a magnetic field. Poyntings Theory shows there is only an electric field. Ampere’s law is not valid.” … “In EQS only the electric field is associated with energy” (Castellenos 1998). EQS involves capacitance features. capacitance is a function only of the geometry of the design and the permittivity of the dielectric material between the plates of the capacitor (Castellenos 1998).
The Magnetic Limit
In the magnetic limit the motion of a magnetic field (or its time variation) produces an electric field while a time varying electric field does not produce a magnetic field (LeBallac 1972). Physically this describes the situation at the macroscopic level where the balance between negative and positive charges. In the magnetic limit there is no displacement current. There can only be stationary currents and no accumulation of charge. The electric field is “non zero” but has no observable effects. (Rousseaux 2006).
These equations describe where magnetic fields charges move with slow velocity.
Quasi Magnetic maxwell equations (Le Blonde and Le Ballac 1972)
Effectively an electric field (Em) is generated by magnetic sources and a magnetic field (Bm) is generated by a magnetic source. In the magnetic limit the electric field is not a time varying (1/c2)∂tEe ) (i.e. Maxwells part of the equation) and will not create a magnetic field (∇ x Bm) to couple back onto the electric field. (Rosseaux 2006). In other words there is no induction, whereby an electric field is induced, or generated by a changing magnetic field. This equation only allows stationary currents
From an engineering perspective “The MQS regime good approximation in the interior and vicinity of good conductors, which takes Faradays laws into account but neglects displacement currents. In the MQS regime, only stationary currents are allowed and these currents cannot explain time changes in the charge density ρ.” (Kutrtz 2010).
In engineering the electric field is incorrectly concluded not to exist because it has not effect. “The MQS regime includes inductive but not capacitive effects, because only stationary electric fields are allowed and these do not change the charge density. Poyntings Theory shows there is no magnetic field. Ampere’s law is valid. … In MQS only the magnetic field has energy. (Castellenos 1998)
Super luminal Electric and Magnetic Fields
LeBlonde and Le Ballac (1973) warned that defining c → ∞ in the quasi static limits was bound to produce questionable results. Instead the value of c should come from the equations.
In a MKSA system the initially <<independent>> coefficients ε0 µ0 turn out to be related by the formula ε0 µ0 c<sup>2</sup>=1. This is sufficient to show that Maxwell’s equations cannot have a nonrelativistic limit (c → ∞) where ε0 µ0 both remain finite. The possibility of keeping one of them finite, at will, implies the existence of the two Galilean limits. In fact the following prescription yields the <<magnetic>> limit: express the relativistic theory in terms of the usual E and B keeping µ0 but eliminating ε0 then let c go to infinity. To obtain the <<electric>> limit express the relativistic theory in terms of E keeping ε0 but eliminating µ0. – Le Blond and Le Ballac 1973
In otherwords if where ε0 < 1 then superluminal fields in the electric limit, and where µ0 < 1 then superluminal fields in the magnetic limit.
The Darwin Limit
A third hybrid limit called the Darwin limit has also been shown to exist for higher velocity charges. The Darwin limits neglects the transverse, but not longitudinal, electric field
“for some problems, e.g., the simulation of charged particles when no high frequency phenomenon or no rapid current change occurs, it is possible to use some simplified model which approximates Maxwell’s equations and can be solved more economically. The Darwin model is such a simplified model. ” (Liao 2008)
Degond and Raviart (1992) decomposed the electric field E into the sum of its transverse component ET and longitudinal component EL, where ET is divergence free and EL is curl free. The Darwin model is obtained by neglecting ∂Et/∂t. In otherwords the transverse electric field that creates the solenoidal part of amperes law is neglected. In MQS and Darwin there is a contribution to the electric field due to magnetic induction (from ∂B/∂t in Faraday’s law). (Castellenos 1998)
Raviart (1994) used Helmholtz decomposition to show the electric field is composed of a longitudinal field (EL) and transverse (ET) field.

Equation showing the decomposition of the electric and transverse fields in the Darwin limit (Raviart 1994). Where EL is curl free and ET is divergence free
The Helmholtz decomposition then produces the following set of separate equations.

This led to the following conclusion by Bauer (2018). “Prior to Maxwell there were two independent div[ergent] curl systems. In Maxwell’s equations there are two curl div[ergent] systems where the results of one feed into the source of the next. In Darwin there are three div[ergent] curl systems where the results fed into the next one.” – Bauer 2018.
From an engineering perspective “The Darwin model includes both capacitive and inductive effects, but there is no radiation and uses the Coloumb Gauge. No interactions are instantaneous (Larsson 2006). Poytings theorem shows there is both electric and magnetic energy but electric energy only shows the Coloumb part of the field. Within the Darwin model the Biot-Savart law is valid and Ampere’s law is not. ” (Castellenos 1998).
Larson (2006) draws some important conclusions. First that the Darwin limits are able to create feedback and thereby oscillations but not radiation. “An important qualitative difference between EQS and MQS on the one side and Darwin’s model on the other is the possibility of natural resonances in the latter. (Larson 2006) A qualitatively new feature of Darwin’s model (as opposed to MQS or EQS) is the possibility of resonance but there is no radiation and the interactions are instantaneous.
Larson (2006) also concludes that the oscillations could include energy oscillating between electric and magnetic energy. “Since the Darwin model includes both capacitive and inductive phenomena it may in principle be used to model systems where the energy oscillates between the electric and magnetic fields. but the frequency must be low enough not to violate the quasistatic assumption.”,
Finally, Larson (2006) recognised that “The use of two complementary quasistatic models in the same physical system is clearly a complicating feature if we like to model the whole system numerically. We would then have to divide the whole spatial region into EQS and MQS subregions with appropriate continuity conditions at the interfaces. A better alternative may be to use the Darwin model which embrace all the physics contained in EQS and MQS, still being quasistatic.”
Conclusion
It is true to say that electric and magnetic fields in the brain result from slow moving, low energy ions. It is therefore true to say that quasi static electric, magnetic and Darwin quasi static fields probably exist in the brain. It is also true that the “non-casual” fields from the ions have curl reflecting the wavelengths on light transmitted from ions, It is also true to say that the wavelengths of the ions in the brain are similar to the (diametric) geometry of brain cells and could therefore create guided wave modes as explained in previous posts. Importantly these Galilean quasi static fields, could create guided wave modes and could interact.
Further is has been shown (Omelyan 1999 a,b) that complex dielectric functions resulting from the oscillations of water reduces the permeability (ε) in the transverse (T) and longitudinal (L) direction differently in water. In particular the permeability of water in a longitudinal direction is greatly reduced to wavelengths including those of ions in the brain. These oscillations would effect fields in the Darwin limit which also function differently in the longitudinal and transverse direction.
Oscillations in water could provide a means of creating switches, wires and waveguides in water for ion sourced fields. The longitudinal properties of oscillating water and longitudinal fields in Darwinian quasi statics are complimentary. The ability of oscillating water to drastically change permeability means water can change the curl and divergence of quasi static electric and magnetic fields enabling ‘calibrated’ (custom) waveguide and modes.